Antibiotics, which are used in animal husbandry for prevention and treatment of bacterial diseases, are an important class of water pollutants due to their large variety, high consumption, and persistence in the environment. The presence of low concentrations of antibiotics compounds and their transformation products has been detected in treated sewage water, clearly showing that some antibiotics products cannot be eliminated during wastewater treatment [1, 2]. Sulfadiazine [SDZ, 4-amino-N-(2-pyrimidinyl) benzene sulfonamide], a potent antibacterial agent belonging to the sulfonamide class of antibiotics, is widely used as a veterinary and human medicine [3]. After administration to livestock, about 50% of SDZ is excreted through manure as is, and 30% is excreted as an acetyl conjugate [4]. Once SDZ and its metabolites is released to agricultural land, they may interact with different soil components, wash off into surface water, or leach into ground water, where it may enter the food chain and impact the environment and human health [5, 6].
Recently, the degradation of SDZ in water by advanced oxidation processes (AOPs) has been investigated. Temesgen et al. have found that ozonation could be used to effectively remove the sulfonamides from water [7]. Premasis Sukul et al. have studied the photolysis of SDZ and identified 6 photoproducts, establishing a tentative reaction pathway. His research also showed that photolysis of SDZ in aqueous solution under simulated sunlight followed first-order kinetics [8]. Carolina Baeza et al. have studied the transformation kinetics of SDZ in lowpressure UV photolysis and UV/H2O2 advanced oxidation processes [9]. The removal of SDZ was also investigated in Fenton and photo-Fenton, TiO2 photo catalysis, photochemical oxidation, and electro-oxidation. Promising results have been achieved using AOPs which are based on in situ generation of strong oxygen-based oxidizers: hydroxyl radicals, ozone, atomic oxygen, hydrogen peroxide, or per hydroxyl radicals, which promote destruction of the target pollutant until mineralization [10]. Plasma as one of the AOPs has been also investigated as a possible method for water treatment. Plasma generated in electrical discharges in liquid or at the gas-liquid interface leads to the formation of oxidizing species: radicals (H·, O·, HO·) and molecules (H2O2, O3, etc.) [11], which are effective for the removal of pollutants. The plasma can be created either directly in the liquid, or in the gas above the liquid, or, both in liquid and in gas. The removal of antibiotics compounds using plasma has been recently studied by several authors [12, 13, 14]. But to the authors’ knowledge the degradation of SDZ by water falling film dielectric barrier discharge together with the study of the resulting degradation products and the proposed evolutionary process has not been investigated up to now.
In this research, SDZ in aqueous solution was removed by water falling film dielectric barrier discharge. The factors affecting the degradation efficiency of SDZ were investigated, and the presences of radical scavengers on the reaction were also studied. At the same time, the 8 degradation intermediates were identified by LC-MS and the degradation mechanism and pathway of SDZ in water by falling film dielectric barrier discharge was focused.
SDZ (analytical standard, purity grade, 99.9%), was purchased from Aladdin Inc. All the chemicals used in the analysis were highperformance liquid chromatography (HPLC) grade. Other chemicals were all analytical grade and used without further purification. H2SO4 and NaOH were used to adjust the pH value of the solution and FeSO4⋅7H2O was used as the source of Fe2+.
The schematic diagram of the reactor is shown in Fig. 1. The cylinder reactor was comprised of a quartz tube with respective inner and outer diameters of 6 nm and 25 mm and a 5 mm aluminum rod which was inserted into the inner tube. The outer electrode was made of aluminum foil glued on the outside of the quartz tube with a length of 150 mm. The SDZ solution was circulated by a peristaltic pump with a flow rate of 50 mL/min and was made to flow as a film on the inner surface of the outer quartz tube. The air was naturally introduced into the reactor, not by any other aerodynamic devices. A pulsed high voltage source (Nanjing SuMan Electronics Co., Ltd., China) was applied to the two aluminum electrodes, which could be operated at an adjustable amplitude voltage. The space between the inner and outer quartz tubes was the electrical discharge area. To operate the falling film reactor, water flowed down over the inner surface of outer quartz tube, making a thin dielectric film. Then, discharges occurred at the interface of the gas and the solution. For every experiment, 100 mL solutions were added into the reactor.
![]() |
Download:
|
Fig. 1.Experimental setup. |
An HPLC system (Agilent, USA, 1200 Series) equipped with a Thermo C18 column (4.6 mm × 250 mm i.d., 5 μm, USA) and a multiple wavelength UV diode array detector was used to analyze the concentration of SDZ. The mobile phase consisted of 70% methanol and 30% water, the flow rate was 1.0 mL/min, and the injection volume was 20 μL. The column temperature was kept at 25 ℃ and a UV detector was set at 265 nm.
SDZ and its degradation products were analyzed by LC-MS (Agilent 1290 Infinity LC/6460 QQQ MS, USA) with an Agilent Eclipse XDB-C18 HPLC column (150 mm × 2.1 mm i.d., 5 μm, Agilent, USA). The mobile phase was a mixture of 50% acetonitrile and 50% water with a flow rate of 0.2 mL/min. The capillary temperature was set to 200 ℃, the spray voltage was 4500 V, and the sheath gas flow rate was 18 arb. The spectra were acquired both in the negative ion scan mode and positive ion scan mode, over the m/z range from 50 to 600.
Total organic carbon (TOC) was determined on a Shimadzu 5000A TOC analyzer. The conductivity of the solution was measured by a DDS-11 AW conductivity meter. UV-vis absorption spectra of the SDZ solutions were measured by a UV-5600PC (Shanghai metash instruments Co., Ltd., China). The pH value of the solution was measured by a pH monitor (Shanghai Kangyi Instrument Co., Ltd., China, PHS-3B). The concentration of H2O2 was determined spectrophotometrically [15].
![]() |
Download:
|
Fig. 2.The factors affecting the degradation efficiency of SDZ: (a) output power intensity (C0 = 10 mg/L), (b) pH value (C0 = 10 mg/L, output power = 100 W), (c) initial concentrations (output power = 100 W), (d) Fe2+ concentrations (C0 = 10 mg/L, output power = 100 W). |
The factors affecting the degradation efficiency of SDZ are shown in Fig. 2. The Fig. 2(a) shows the degradation of SDZ under different output power intensity. The output power has a significant effect on the active species production. The degradation efficiency of SDZ increased with increasing output power intensity. The maximum degradation efficiency of SDZ was 96% after 15 min under the condition of 150 W, while the degradation efficiencies were 87% and 94% in the case of 100Wand 120 W, respectively. As the output power increases, electrons gain more energy in the electric field and induce more ionization of oxygen and water molecules by collision [16], which increased the amount of the active species (HO·, O·, H·, H2O2, O3). Based on the above results, the degradation amount of SDZ was increased.
Most antibiotics have acidic and/or basic functionalities; their ionization state is controlled by both pH and their acid dissociation constant (pKa) [17]. The pKa of SDZ are: pKa1 = 1.57 and pKa2 = 6.50, which mean that SDZ has a neutral form at 1.57 < pH < 6.50 and an anionic form at pH > 6.50. Fig. 2(b) shows the effect of the pH value on the degradation efficiency of SDZ and indicated that the degradation efficiency was the highest under weak alkaline conditions (pH = 9.10),where SDZwas in its anionic form. Researchers have reported that the major active species involved in the degradation of organic pollutants using gas discharge were HO·, O3 and H2O2, and under strongly alkaline conditions the generated HO· in the discharge reacted with carbonate ions immediately, which decreased the amount of HO· substantially [18], thus pH 11.04 is not suitable for the degradation of SDZ.
As seen in Fig. 2(c), the degradation efficiency of SDZ decreases with increasing concentration. The SDZ and its intermediates are mainly oxidized by active species generated in discharge. When more SDZ is present in the solution, the active species are consumed not only in reactions with SDZ, but also in reactions with the intermediates. As such, when the inner concentration is higher, there is strong competition for reaction with the active species between SDZ and its intermediate products. In this case, the concentration of active species is probably not high enough to fully oxidize SDZ and its intermediates.
In the previous research, many researchers have found that the degradation efficiency of organic contaminants under the condition of pulsed discharge could be improved by the Fe2+ [19]. Fe2+ was added to the treated solution to increase the oxidizing power of generated H2O2 by the production of HO· from the Fenton reaction [20]:
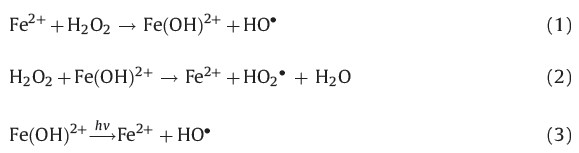
Fig. 2(d) shows the effect of adding different concentrations of Fe2+on degradation efficiency of SDZ. It is shown in Fig. 2(d) that in the range of 0-1.0 mmol/L the degradation efficiency of SDZ increased with increasing Fe2+ concentration, which was contrary to the results from the range of 1.0-2.0 mmol/L. The lower enhancement of degradation efficiency at higher Fe2+concentration could be explained by the competition between Fe2+and the organic substance for HO·, with a reaction as follows [21]:

Excessive Fe2+ consumes HO·, reducing the amount available to react with SDZ, decreasing the degradation efficiency.
The variation of pH values of the solution during the treatment process is shown in Fig. 3 for all three initial pH values for SDZ. The pH value of the solution decreased rapidly during the first 3 min and then stayed around 1.40 for the last 15 min. The curves also show that the conductivity considerably increased linearly for all three initial pH values for SDZ, which means the ion concentration of the solution increased. The decrease of the pH value, accompanied by the increase of the conductivity, might be due to the formation of H3O+ ions and several special acidic substances such as nitric acid and nitrous acid during discharge [16]. Also, the formation of NO3- and NO2- from the dissolution of nitrogen oxides such as reactions (5)-(11) should be responsible for the decrease of the pH value and the increase of the conductivity. In addition, the formation of H2SO4 formed from the degradation of SDZ decreases the pH value as discussed in the Section 3.5.
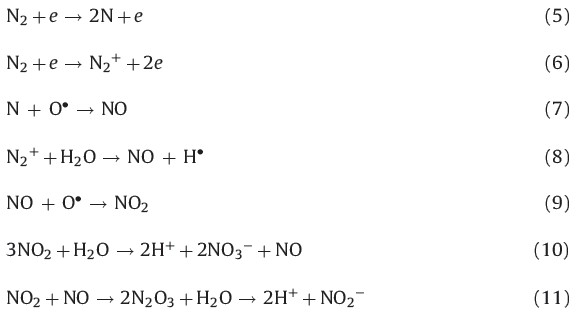
![]() |
Download:
|
Fig. 3.The change of pH values and conductivity during the treatment (C0 = 10 mg/L, output power = 100 W). |
Fig. 4 shows the effect of adding different radical scavengers on the degradation efficiency of SDZ. Sodium carbonate, iso-propyl alcohol and tert-butyl alcohol are all hydroxyl radical scavengers, which could scavenge hydroxyl radicals in aqueous solution, and consume the amount of hydroxyl radical immediately [22]. The SDZ degradation efficiency was considerably reduced upon the addition of 150 mg/L sodium carbonate, 150 mg/L iso-propyl alcohol and 150 mg/L tert-butyl alcohol. With an addition of isopropyl alcohol, the extent of degradation of SDZ decreased more drastically than from tert-butyl alcohol and sodium carbonate. The reaction rate constant (k) of iso-propyl alcohol, tert-butyl alcohol and sodium carbonate with hydroxyl radical are 1.9 × 1010 mol L-1 s-1, 6.0 × 108 mol L-1 s-1 and 3.9 × 108 mol L-1 s-1, respectively [22], thus the reaction speed of iso-propyl alcohol with hydroxyl radical is highest, leading to the most severe decrease in the degradation of SDZ. In the presence of hydroxyl radical scavengers, the degradation efficiency was inhibited, but not completely, indicating that ·OH played a primary role in the reaction, but not the only role.
![]() |
Download:
|
Fig. 4.Effect of addition of radical scavengers (C0= 10 mg/L, output power = 100 W). |
![]() |
Download:
|
Fig. 5.Change of TOC removal (C0 = 10 mg/L, output power = 100 W). |
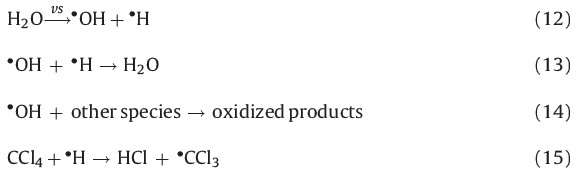
In contrast with adding hydroxyl radical scavengers which considerably reduced degradation efficiency, Fig. 4 indicated that the SDZ degradation efficiency was remarkably enhanced with CCl4 addition. CCl4 is one of the hydrogen radical scavengers which could react with hydrogen radical immediately. Reactions (12)- (15) indicate the enhancement mechanism of CCl4 for the degradation of SDZ. Hydrogen radical in the system were scavenged with CCl4 (reaction (15)), and reduce the chances of their recombination with hydroxyl radical (reaction (13)), thus increasing the concentration of hydroxyl radicals.
The total concentration of organic pollutants in aqueous solution can be shown through the change of the TOC value, which could also reflect the mineralization rate of the pollutants in the solution. As shown in Fig. 5, only 25% TOC was removed at 30 min, indicating slower mineralization being obtained, while about 98% degradation efficiency of SDZ was achieved. It was obvious that only a small part of SDZ was mineralized. This may be explained by the generation of intermediates degrading from SDZ, which resist further degradation and react with hydroxyl radicals. The change in the UV-vis absorption spectra of SDZ solution during reaction processes has been shown in Fig. 6. After treatment, the absorbance at 265 nm shows a decrease, which indicated the degradation of SDZ molecules. However, the absorbance at around 200-250 nm shows an abrupt increase in contrast to that at 265 nm, which indicates that the formation amounts of intermediates increased with reaction times. This suggests that the mineralization of these intermediates is a much slower and difficult process.
![]() |
Download:
|
Fig. 6.UV–vis spectrum along with reaction time (C0 = 10 mg/L, output power = 100 W). |
The extensive LC-MS has been successfully used for the identification of SDZ and its degradation intermediates by other researchers [8]. Based on the mass spectra, 8 intermediates of SDZ were detected, which in the Table 1. The identification of the structures corresponding to these fragments led to the degradation pathway during the reaction process depicted in Scheme 1.According to Boreen et al., the potential cleavage sites of sulfonamides are as follows [23]:
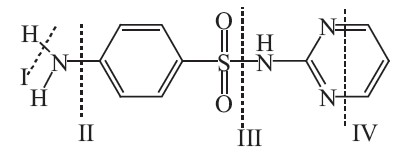
![]() |
Table 1 The degradation intermediates of SDZ. |
![]() |
Download:
|
Scheme 1.The degradation pathway of SDZ under plasma treatment. |
The water falling film dielectric barrier discharge was investigated with the aim of the removal of SDZ in water. The water falling film dielectric barrier discharge was very effective in the degradation of SDZ; the inner concentrations of 10 mg/L SDZ can be all removed within 30 min. The parameters that affect the degradation of SDZ such as output power intensity, pH value, inner concentration and Fe2+ were all strongly affected the SDZ degradation efficiency. The formation of H3O+ ions, organic acids, NO3-, NO2- and SO42- led to the decrease of the pH value and the increase of conductivity. In the addition to hydroxyl radical scavengers, the degradation of SDZ was inhibited, indicating that ·OH plays a primary role in the reaction. It was also found that the degradation of SDZ and the TOC removal was greatly enhanced by hydrogen radical scavenger CCl4, which was due to the reaction of CCl4 with ·H that reduce the chances of their recombination with ·OH. But, the results obtained with the change of TOC indicated that the intermediates were quite refractory to further mineralization, leading to ineffective mineralization. Based on the 8 intermediate products identified by LC-MS, the proposed degradation mechanism was investigated, and the degradation pathway of SDZ involved a series of hydroxylation, oxidative and opening of the ring processes. The ·OH was the major active species and played an important role in the degradation of SDZ.
This research is supported by the State Key Laboratory of Pollution Control & Resources Reuse.
[1] | A.G. Trovo, S.A.S. Melo, R.F.P. Nogueira, Photodegradation of the pharmaceuticals amoxicillin, bezafibrate and paracetamol by the photo-Fenton process application to sewage treatment plant effluent, J. Photochem. Photobiol. A: Chem. 198 (2008) 215-220. |
[2] | M.J. Benotti, B.D. Stanford, E.C. Wert, et al., Evaluation of a photocatalytic reactor membrane pilot system for the removal of pharmaceuticals and endocrine disrupting compounds from water, Water Res. 43 (2009) 1513-1522. |
[3] | A.B.A. Boxall, L.A. Fogg, P.A. Blackwell, et al., Veterinary medicines in the environment, Rev. Environ. Contam. 180 (2004) 1-91. |
[4] | M. Lamshöft, P. Sukul, S. Zühlke, et al., Metabolism of 14C-sulfadiazine after administration to pigs, Anal. Bioanal. Chem. 388 (2007) 1733-1745. |
[5] | P. Sukul, M. Spiteller, Sulfonamides in the environment as veterinary drugs, Rev. Environ. Contam. Toxicol. 187 (2006) 67-101. |
[6] | X.P. Hong, J.Y. Ma, Electrochemical study of sulfadiazine on a novel phthalocyanine-containing chemically modified electrode, Chin. Chem. Lett. 24 (2013) 329-331. |
[7] | T. Garoma, S.K. Umamaheshwar, A. Mumper, Removal of sulfadiazine, sulfamethizole, sulfamethoxazole, and sulfathiazole from aqueous solution by ozonation, Chemosphere 79 (2010) 814-820. |
[8] | P. Sukul, M. Lamshoft, S. Zuhlke, et al., Photolysis of 14C-sulfadiazine in water and manure, Chemosphere 71 (2008) 717-725. |
[9] | C. Baeza, D.R.U. Knappe, Transformation kinetics of biochemically active compounds in low-pressure UV Photolysis and UV/H2O2 advanced oxidation processes, Water Res. 45 (2011) 4531-4543. |
[10] | S. Esplugas, D.M. Bila, L.G.T. Krause, et al., Ozonation and advanced oxidation technologies to remove endocrine disrupting chemicals (EDCs) and pharmaceuticals and personal care products (PPCPs) in water effluents, J. Hazard. Mater. 149 (2007) 631-642. |
[11] | P. Lukes, M. Clupek, V. Babick, et al., Generation of ozone by pulsed corona discharge over water surface in hybrid gas-liquid electrical discharge reactor, J. Phys. D: Appl. Phys. 38 (2005) 409-416. |
[12] | D. Gerrity, B.D. Stanford, R.A. Trenholm, et al., An evaluation of a pilot-scale nonthermal plasma advanced oxidation process for trace organic compound degradation, Water Res. 44 (2010) 493-504. |
[13] | H. Krause, B. Schweiger, J. Schuhmacher, et al., Degradation of the endocrine disrupting chemicals (EDCs) carbamazepine, clofibric acid, and iopromide by corona discharge over water, Chemosphere 75 (2009) 163-168. |
[14] | M. Magureanu, D. Piroi, N.B. Mandache, et al., Degradation of pharmaceutical compound pentoxifylline in water by non-thermal plasma treatment, Water Res. 44 (2010) 3445-3453. |
[15] | C. Korman, D.W. Bahneman, M.R. Hoffman, et al., Photocatalytic production of H2O2 and organic peroxides in aqueous suspensions of TiO2, ZnO, and desert sand, Environ. Sci. Technol. 22 (1988) 798-806. |
[16] | Y. Zhang, M.H. Zhou, X.L. Hao, Degradation mechanisms of 4-chlorophenol in a novel gas-liquid hybrid discharge reactor by pulsed high voltage system with oxygen or nitrogen bubbling, Chemosphere 67 (2007) 702-711. |
[17] | P. Sukul, M. Lamshöft, S. Zühlke, et al., Sorption and desorption of sulfadiazine in soil and soil-manure systems, Chemosphere 73 (2008) 1344-1350. |
[18] | A.T. Sugiarto, T.M. Ohshima, T. Sato, et al., Oxidative decoloration of dyes by pulsed discharge plasma in water, J. Electrostat. 58 (2003) 135-145. |
[19] | X.L. Hao, M.H. Zhou, Q. Xin, et al., Pulsed discharge plasma induced Fenton-like reactions for the enhancement of the degradation of 4-chlorophenol in water, Chemosphere 66 (2007) 2185-2192. |
[20] | Y.F. Sun, J.J. Pignatello, Photochemical reactions involved in the total mineralization of 2, 4-D by Fe3+/H2O2/UV, Environ. Sci. Technol. 27 (1993) 304-310. |
[21] | B.S. Lodha, S. Chaudhari, Optimization of Fenton-biological treatment scheme for the treatment of aqueous dye solutions, J. Hazard. Mater. 148 (2007) 459-466. |
[22] | G.V. Buxton, C.L. Greenstock, W.P. Helman, et al., Critical review of rate constants for reactions of hydrated electrons, hydrogen atoms and hydroxyl radicals (·OH/O·-) in aqueous solution, J. Phys. Chem. Ref. Data 17 (1988) 513-886. |
[23] | A.L. Boreen, X.A. Amold, K. McNeill, Photochemical fate of sulfa drugs in the aquatic environment: sulfa drugs containing five-membered heterocyclic groups, Environ. Sci. Technol. 38 (2004) 3933-3940. |
[24] | W.R. Haag, C.C. Yao, Rate constants of reaction of hydroxyl radicals with several drinking water contaminants, Environ. Sci. Technol. 26 (1992) 1005-1013. |
[25] | C.C. Yao, W.R. Haag, Rate constants for direct reaction of ozone with several drinking water contaminates, Water Res. 25 (1991) 761-773. |
[26] | Z.B. Guo, F. Zhou, Y.F. Zhao, et al., Gamma irradiation-induced sulfadiazine degradation and its removal mechanisms, Chem. Eng. J. 191 (2012) 256-262. |